Enter the void
But the atomists didn’t get by without being challenged. The philosopher Parmenides viewed things very differently. According to him, nothingness was a mere abstract concept. The universe was actually filled to the brim with a substance called plenum, and no matter where you looked, you would never find a space that lacked this substance.
Aristotle notably sided with Parmenides and added a new type of substance, which he called the aether, to explain how celestial bodies moved through the heavens.
Then, in 1905, Albert Einstein shook things up with two separate discoveries. His theory of special relativity showed that light could in fact travel through empty space without any need for an infinite aether to transport it.
Second, he conclusively demonstrated that atoms of finite size were real — a concept that was still in question at the time — by mathematically describing the movement of particles suspended in water.
Both of these discoveries placed limitations on what could or could not be defined as nothing. Let’s start with the limited size of atoms. Leucippus and Democritus would doubtless have felt vindicated by Einstein.
But things get tricky when it comes to size, of course. Physicists in the 19th and 20th centuries made the crucial discoveries that atoms were actually made of protons, neutrons, and electrons.
Protons and neutrons were thought to be indivisible for a while, until physicists began shooting subluminal electrons at them in underground, mile-long particle accelerators. This causes atoms to explode and allows physicists to sort through the sub-atomic debris. Because of this work, we now know that protons and neutrons are actually Kinder eggs full of quarks.
That’s okay…maybe quarks are the smallest things that exist?
Unfortunately, some very sharp physicists — armed with sophisticated mathematics — demonstrated that quarks might actually be made of tiny vibrating strings.
Could there be anything smaller than a string? Since, in order to talk about strings, you must first invoke the existence of eleven separate dimensions, we’ll just say it’s complicated.
But you can still zoom in further. If you shrink down to the mathematically smallest measurable scale, called the Planck length, you might find yourself surrounded by miniature black holes. Don’t bother asking if there’s anything smaller than the black holes. You won’t like the answer.
At this point, you might be ready to throw in the towel. We’re unlikely to figure out the nature of nothingness by peering increasingly closer at the building blocks of reality. Physicists genuinely don’t know what the smallest thing in our universe is, or if there even is such a thing. Carl Sagan poetically echoed the theory of an infinitely recursive universe in the book and show, “Cosmos.”
“There is an idea — strange, haunting, evocative — one of the most exquisite conjectures in science and religion. It is entirely undemonstrated; it may never be proved. But it stirs the blood. There are, we are told, an infinite hierarchy of universes, so that an elementary particle, such as an electron, in our universe would, if penetrated, reveal itself to be an entire closed universe. Within it, organized into the local equivalent of galaxies and smaller structures, are an immense number of other, much tinier elementary particles, which are themselves universes at the next level, and so on forever.”
But maybe size doesn’t matter. Leucippus and Democritus were wrong about atoms not having any empty space inside them. In some ways, we can think of atoms as being made of mostly empty space with just a dash of matter to give them flavor. What if we took a close look at that emptiness to see if it contains something or nothing?
In the smallest atom, hydrogen, a single proton is distantly orbited by a lone electron roughly 5.3 X 10-11 m away. If a proton ballooned to the size of your head, it’s nearest electric partner would reside at a distance of about two miles. That’s plenty of room to go poking around for nothing.
Except, in quantum physics, electrons never occupy a single space at a given time. Rather, they are in a superposition of every possible location around the atom’s nucleus, until you look at them, and they furtively pick a single position. So the empty space within atoms is not really empty. Instead — because quantum mechanics is weird — it’s a vague expanse of potentiality with fuzzy borders that extend to the edge of the observable universe.
You could try freezing atoms!
If you cooled atoms all the way down to absolute 0 degrees Kelvin, that should get the electrons to stop moving, allowing you to inspect the nothingness between them. But here, quantum mechanics throws us another curve ball.
According to Werner Heisenberg, and a theory he developed called the uncertainty principle, the more you know about a particle’s position, the less you know about its velocity. This is not due to the limitations of scientific instruments, but is instead thought to be an inherent property of matter and energy.
If you could completely freeze an atom, you could determine exactly where its electrons, protons, and neutrons were located, which would mean their velocities would be completely unknowable. Even at the coldest temperatures experimentally achieved in the lab, physicists still record a faint heat from the potential — but not quite actual — velocities of all the constituent particles inside an atom.
We don’t have to restrict ourselves to atoms, though. Let’s do away with them altogether by creating a square-inch-sized vacuum with no particles at all. Surely there would be nothing inside?
Not according to physicist Paul Dirac. You’ll recall that Einstein did away with the idea that an aether permeated the universe, but this isn’t entirely true. Instead, the aether was replaced by a similar medium with a few hardboiled differences.
Dirac postulated the existence of quantum fields, which, like the aether, suffuse the cosmos. According to quantum field theory, everything — both atoms and the space around them — is made of fields. For each elementary particle that we know of — electrons, quarks, neutrinos, etc. — there is a corresponding field that gives rise to them. The fields are considered to be quantum because they can only manifest themselves in discrete quantities, or quanta. The ground state for many of these fields is zero, which corresponds to a space with “no” particles (the notable exception being the Higgs field. If you want to know why, there’s fun sombrero visualization that helps explain it).
An absence of particles sounds promising! Even if the world is permeated with fields, perhaps those fields are perforated with emptiness. You probably know where this is going…
Quantum field theory has one last trick up its sleeves to subvert out expectations, and again, we have Heisenberg to thank for it. Not only can we not measure a particle’s momentum and position simultaneously, it turns out that time and energy also have a similarly inverted relationship. The more you know about how much energy a particle has, the less you know about how long the particle will remain in that energetic state. Again, this is not due to a limitation in scientific instruments but instead is an inherent property of matter and energy. This means the longer a particle remains in a particular state, the more precisely you can measure its energy.
Take a hydrogen atom, for example, which has one proton, one neutron, and one electron. Hydrogen is fairly stable, meaning it sticks around in the same form for a long time; most of the hydrogen around today was formed seconds after the birth of our universe. That means you can precisely measure the amount of energy stored in its subatomic particles.
On incredibly small time scales, on the order of a septillionth (1024) of a second, the less certain you can be about how much energy a particle or a field contains. If you look closely at our square inch of empty space, you’ll see that what you mistook for nothing is actually a roiling sea of what physicists call virtual particles.
In a span of time so brief that even the universe seems unable to register it, virtual particles briefly materialize from nothing and disappear back into nothing. The process occurs so quickly that it doesn’t technically violate the first law of thermodynamics, the one that says energy can neither be created nor destroyed.
We have to be careful here, however. Virtual particles aren’t real, in the sense that they don’t stick around and cannot be directly observed. In a way, they’re merely a mathematical tool to describe something for which we don’t seem to have an analogue up here in the macro world. But whatever virtual particles are or are not, you and I observe them indirectly all the time. Virtual photons are the reason we have electricity in our homes and magnets on our fridge. Virtual gluons hold quarks together, which allows for the formation of atoms, molecules, and planets. Virtual W and Z bosons spark the radioactive decay that powers our sun. An as-of-yet unknown virtual particle might mediate gravity.
In any case, it would seem that we’ve hit a wall. No matter where or how hard we look, we seem incapable of finding a discrete space that can be defined as something and another space that is devoid of that something. Instead, both seem to be polar but unified properties of a grand whole, which is us. Einstein put it this way, “We may therefore regard matter as being constituted by the regions of space in which the field is extremely intense… There is no place in this new kind of physics for both the field and matter, for the field is the only reality.”
Down here, at the subatomic scale where time no longer has any meaning we’re capable of comprehending, there is one additional property of matter that helps clarify what our universe of stuff is made of and creates the equally perplexing mystery of why we have a universe of stuff at all.
In the process of formulating the first iteration of quantum field theory, Paul Dirac hit a snag. Dirac was exclusively modeling electrons, and the math he’d derived beautifully and accurately described their behavior, but it would only do so if he presupposed the existence of an entirely new type of particle, one with the exact same properties (mass, spin) as an electron, but a positive rather than negative electric charge.
Instead of throwing out the math and starting from scratch, Dirac left the new type of matter in his equations and concluded that the universe contained within it a type of matter that physicists had been totally unaware of up to that point. Four years later, positrons were indirectly observed in a bubble chamber, verifying his prediction. Scientists had discovered anti-matter.
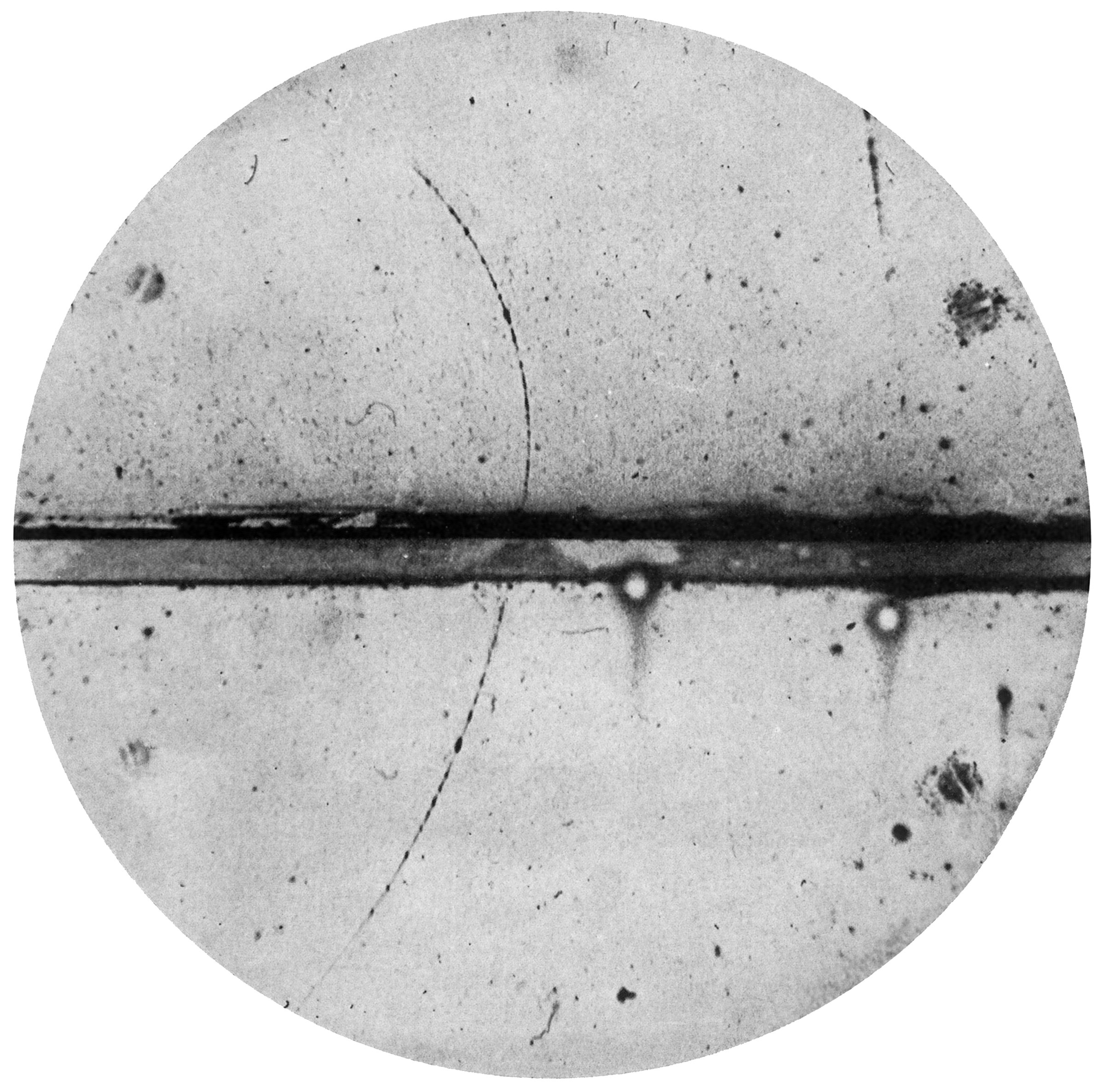
Matter and anti-matter are also always created in equal amounts. Virtual particles with mass and charge, for example, always arise from the void in matter/anti-matter pairs. In fact, there theoretically should have been equal amounts of matter and anti-matter created in the first moments of the universe. If that had been the case, all of it would have combined and annihilated, leaving behind nothing but energy. Since we live in a universe of stuff, we can be fairly confident this didn’t happen, but why is that the case?
There are other, mathematically equivalent, ways to think of anti-matter that can help answer this question. On our way to discussing them, we’ll swim back to the shallows with the help of a fictional girl with synesthesia and the prints of another local artist with a passion for Florida’s wild spaces.